Keywords: Microcalorimetry, electrochemical, heat, battery, Li-ion, primary, secondary, self-discharge, parasitic reaction.
MC158
Background
Electrical energy powers our lives as it can readily be converted into heat, light, chemical bonds, or mechanical work whenever we need it. More and more applications require the electricity be taken “off-grid” as in cell phones, vehicles, and various household appliances. Many of these devices requires large quantities of energy stored in compact spaces. This has led to extensive innovation and development of battery technologies with focus on extending charge cycles of rechargeable cells, usable service life, and safety. It is not an overstatement that the Li-ion battery in its various chemistries and shapes is key for the development of battery powered vehicles and various portable devices. Indeed, the inventors of the Li-ion cell were rewarded the 2019 Nobel prize in chemistry with the motivation “they created a rechargeable world”. Different cell chemistries are being explored while the Li-ion cells currently dominates R&D activities and the market for rechargeable batteries.
The electrochemical processes that take place in batteries, whether under load or charging conditions give rise to heat exchange with the surroundings. The work performed as charged species flow internally in a cell gives rise to heat generation as well as the redox processes at the anode and cathode and various parasitic side reactions that are responsible for limiting the service life of a battery.
Processes causing changes in matter are generally accompanied by an exchange of heat with its surroundings. The faster the rate of a chemical or physical change, the higher the heat generation rate. Isothermal calorimetry is today an established technique for gaining insight into a wide range of physical, chemical, or biological phenomena. In an isothermal microcalorimeter the heat evolution is monitored continuously in real time while the measured specimen is kept at a constant temperature.
Isothermal calorimetry has been used over several decades to monitor self-discharge in predominantly primary batteries such as those used in pacemakers, Hansen and Hart (1978). Over the recent decade a strong increase in the interest of isothermal microcalorimetry has been observed in battery research and development, both in academia and industry. This increased activity has to a large extent focused on secondary batteries, especially Li-ion cells.
The type of microcalorimeters generally used are of the heat conduction or heat flow type with removable sample vessels containing the battery specimen under study, see Suurkuusk et al (2017) for a detailed description of a modern microcalorimetric system. The calorimeters are mounted in a highly regulated environment with temperature stability in the µK scale. As heat is produced or absorbed by a sample the thermal energy flow between the sample and the thermostat environment, keeping the sample temperature constant. The heat flow is measured by thermoelectric sensors placed between the sample and its temperature regulated surroundings.
The aim of this note is to give an overview of isothermal microcalorimetry in battery research, development and quality control in order to illustrate the versatility and providing an idea of the possibilities of isothermal microcalorimetry.
Heat Production and Battery Processes
When a battery is charged or discharged, heat is produced due to a variety of heat producing/consuming events. Dahn et al (1985) studied intercalation of Li to LixMo6Se8 in an electrochemical cell by isothermal calorimetry. They provided a model that considered the various contributions to the total heat production for a rechargeable Li-ion cell simply illustrated by the following terms.
PTotal = PPolarization + PEntropic + PParasitic (1)
Their work aimed at elucidating the reversible entropic term to experimentally estimate the change in entropy of the intercalation compound as a function of the lithium load, x. The purpose of their work was to experimentally validate theoretical gas-lattice models for intercalation.
The polarization term, which is exothermic, includes any process responsible for deviation of the cell voltage relative to the thermodynamic voltage. An example is the flow of charged species like electrons or ions within the cell. The reversible term constitutes the entropy change of the cell during charging or discharging, e.g., changes of the thermodynamic state of an electrode material with the number of intercalated Li-ions. Parasitic reactions are generally irreversible changes of the cell chemistry that limits the battery service life. The amount of heat produced due to such reactions is a direct measure of the extent to which they limit the service life of a battery.
The model was later used to rationalize a method for quantification of parasitic reactions in Li-ion cells by use of isothermal microcalorimetry, Krause et al (2012).
Self-Discharge
Batteries under open circuit conditions tends to generate low level heat flows due to self-discharge processes. Self-discharge may be defined as loss of energy which reduces the usable service life of a battery. While this is relevant for primary batteries, secondary batteries also undergo self-discharge which, in part, is irreversible and in part reversible when recharging the cell. Although the mechanisms of self-discharge processes are not very well understood for many cell types it is a reasonable assumption that the higher the heat generation rate for similar battery types, the shorter is the shelf-life or recharge cycle of the battery.
Figure 1 shows self-discharge heat flow and cumulative heat curves for commercial AAA NiMH batteries from two different manufacturers. The batteries were charged to 100% before introduced to the calorimeter at 25 ºC. it is seen that battery 1 shows a higher discharge rate during the initial 3 days where after the heat flow from the two batteries ends up at approximately the same level. The difference in released energy between the two batteries after 5.7 days is just under 100 joules as revealed from the cumulative heat curves.
Roth (1999) studied self-discharge of Li-ion cells as a function of temperature, and state of charge (SOC) with two different cathode intercalation metal-oxide compounds. Strong dependency of the self- discharge processes was found on SOC in the range 0-100% and temperature in the interval 40-70 ºC. The microcalorimetric measurements revealed higher reactivity of cells with LixNi0.8Co0.2O2 compared to LixCoO2 cathodes. In addition, correlation was found between the propensity of thermal runaway and the measured heat flow in the studied temperature interval.
Hansen and Hart (1978) characterized internal power losses of pacemaker batteries with the purpose to introduce a quick and reliable quality assurance method for eliminating batteries with abnormally high self-discharge rates. They successfully distinguished batteries of high and low self-discharge rates by testing MgO/Zn, and Li-iodine cell types. They also noted that the battery age influences the self-discharge heat flow that need to be weighed in when interpreting the calorimetric data.
Today, pacemaker batteries can be quality tested in an isothermal microcalorimeter, these tests are often performed on the medical device i.e., the pacemaker itself. Heat flow from a passive device should be very low, below 20 µW and more often below 10 µW.

Closed-Circuit Measurements
With a modern microcalorimetric system, like the TAM IV or TAM XL, several experimental setups for measuring battery processes at closed circuit conditions are possible. Specially designed insertion ampoules holding the battery or other electronic specimen permits connection of electrical leads. The wires are taken out from the calorimeter for connection to an externally placed electronic device, e.g., a resistor and/or other electronic device such as a power source, voltmeter etc. Hereby the heat production in the battery can be measured during charge/discharge cycles.
With a multichannel calorimetric platform, like TAM IV, two or more calorimeters can be used simultaneously and independently to measure the heat flow from an electronic device, such as a resistor, in addition to the battery. Such measurements complete the energy budget in term of the production of heat. Figure 3 shows the heat flow and cumulative heat from the measurement of a commercial silver oxide coin cell connected to a 380-ohm resistor placed in a second calorimeter.
As the current through the resistor decreases with time as the voltage drops a concomitant increase of the heat production of the battery is observed from the graphs. When the heat flow of the resistor drops towards zero after approximately 23 hours of measurement the total amount of heat released is about 330 joules corresponding to a residual energy content of 75 mAh.
The term “electrochemical calorimetry” has been adopted to describe the combination of isothermal microcalorimetry with a high-resolution voltage meter and precision current source for studies of charging/discharging cycles at different charge conditions, Krause et al (2012). In addition to correlating calorimetric data with coulombic efficiencies, such experimental setups makes it possible to separate and quantify the different contributions to the total heat flow as per equation 1.
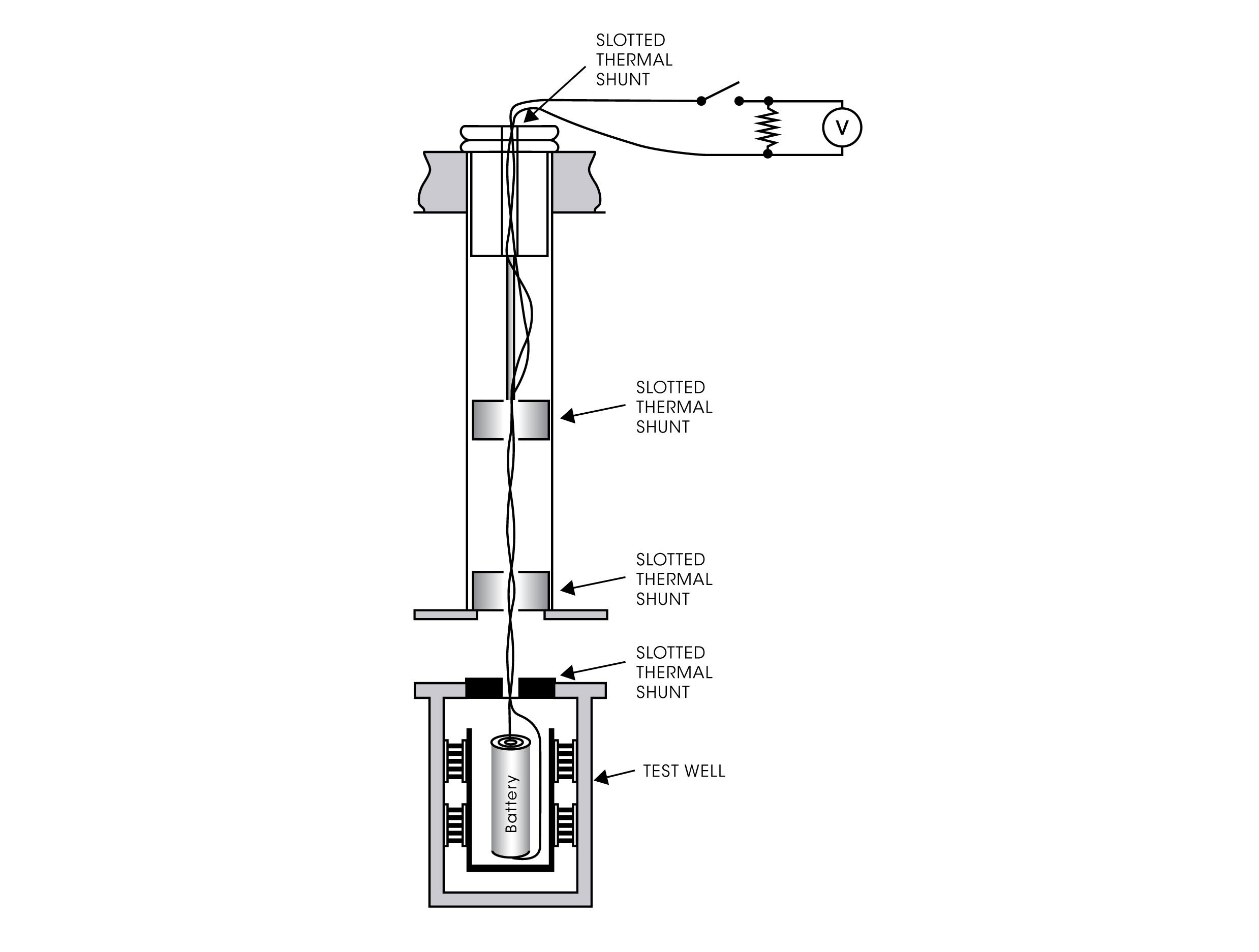

Parasitic Reactions
Krause et al (2012) described a method to separate the parasitic heat events from the total heat production and thus quantify the parasitic reactions. The approach was to measure entire charge/discharge cycles. The contribution from polarization was obtained from the integrated voltage hysteresis and could then be subtracted from the measured heat flow over an entire charge/discharge cycle. By realizing that the reversible heat flow from a cycle cancel out when integrating over the entire cycle the remaining heat is a result of parasitic irreversible reactions after the contribution from polarization effects has been subtracted. They found linear correlations between the parasitic heat and the amount of lost active Li which made it possible to determine the enthalpy change for the parasitic reactions which for this case was estimated to -212 kJ mol-1. The parasitic energy was found to be higher with a graphite electrode of higher surface area compared to one with lower surface.
Downie et al 2013 combined a TAM microcalorimeter with a battery cycler to qualitatively study the effect of an electrolyte additive on the stability of LiCoO2/graphite pouch cells over a defined voltage range. Low current charge/discharge cycles with various amounts of vinylene carbonate keeping the cells identical in other respects were measured. Any differences in the heat flow from the cells containing the additive relative to a control with no additive could then be attributed to the effect of the additive. Clear impact was observed on the heat flow, the higher the concentration the lower the heat flow indicating increased stability. It was further concluded that beyond a certain concentration (2%) the additive had just a minor additional effect on the stability enabling the assessment of optimal concentration of vinylene carbonate in the electrolyte.
Structural Evolutions
During charging, Li ions moves from the positive electrode and intercalate into the negative electrode. This changes the crystal structure, which affects the configurational entropy and can be detected in the heat flow signal, the second term in equation 1. If this is a reversible process the signal from charge/discharge will cancel out. However, configurational entropy sometimes gives a stronger feature in the heat flow signal and this can be correlated with Lithium plating, an event that can be difficult to detect by electrochemical methods. Downie, at al (2013).
Isothermal heat flow data has also revealed unexpected material behaviors as crystallization. Crystallization events gives rise to a very sharp exotherm that is hardly noticeable by electrochemical methods Chevrier et al (2021).
Safety Assessments
Storing chemical energy at high densities is generally accompanied with safety hazards due to the possibility of exothermic decomposition that may lead to runaway reactions resulting in fire or explosion. Just as with storage of high energy materials like explosives this is true for batteries where, for example, efforts are being made to increase the energy content of Li-ion cells in which the lithium in its reduced form is very reactive. The heat production rate in the material is naturally a critical parameter when assessing potential temperature runaway situations. Microcalorimetry is a proven technique for such assessments as applied to a range of high energy materials. In addition to the heat production rate, estimation of the temperature-rise in a battery during various state of charge in charging or recharging situations requires reliable determination of the thermal properties of whole batteries. The heat capacity of the battery is one such property that can be accurately determined by microcalorimetry. Figure 4 shows a typical “step-isothermal” measurement of a whole battery.
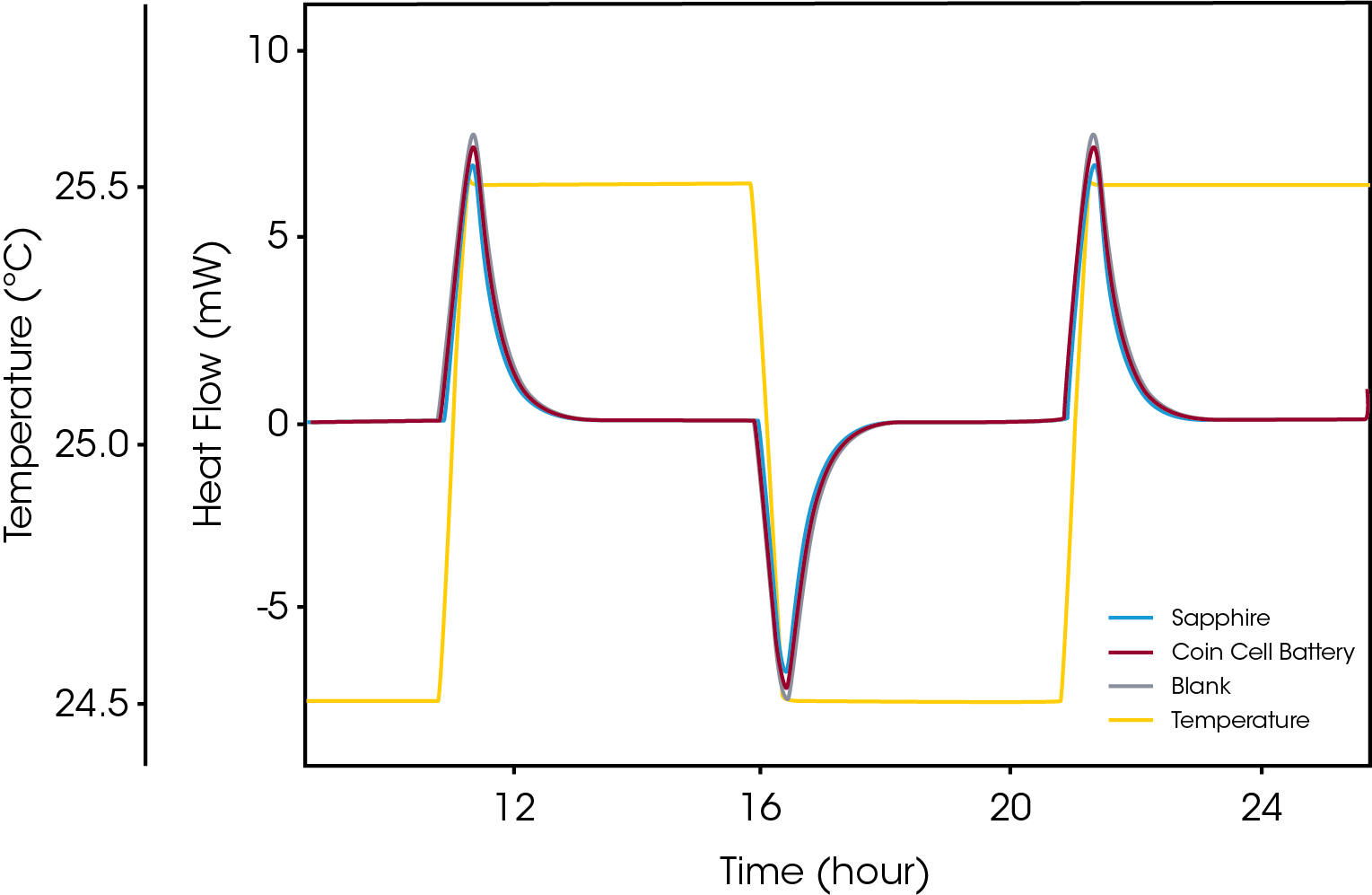
Conclusions
With the development of highly flexible microcalorimetric instruments having detection limits approaching a nanowatt, increased interest for calorimetric measurements is seen in battery research, development, and quality control. With the range of insertion vessels of different shapes and sizes, whole batteries of different shapes, such as coins, cylinders of AA, AAA, and 18650 sizes, and pouches can readily be measured either under open or closed-circuit conditions.
The detection limit of the most sensitive TAM IV calorimeters allows measurements of low self-discharge rates even in the smallest coin shape batteries or heat flow from charge/discharge cycles at very low currents.
In combination with a current source and voltmeter or a commercial battery cycler quantitative determination of parasitic reactions can readily be determined as a function of voltage, temperature, and variable battery chemistry.
The flexibility of the TAM system allows a multitude of experimental setups to be designed and the experimental limits are only up the imagination or creativity of the researcher.
References
1. Chevrier et al (2021) Isothermal Calorimetry Evaluation of Metallurgical Silicon as a Negative Electrode Material for Li-Ion Batteries, J. Electrochem. Soc. 168
2. Downie (2013) The Impact of Electrolyte Additives Determined Using Isothermal Microcalorimetry, ECS Electrochemistry Letters, 2 (10)
3. Downie (2013) In Situ Detection of Lithium Plating on Graphite Electrodes by Electrochemical Calorimetry, Journal of The Electrochemical Society, 160 (4)
4. Hansen, L.D., and Hart (1978) The characterization of internal power losses in pacemaker batteries by calorimetry, J. Electrochem. Soc.: Electrochemical science and technology 125(6).
5. Krause, L. J., Jensen, L. D., and Dahn, J. R. (2012) Measurement of parasitic reactions in Li-ion cells by electrochemical calorimetry, J. Electrochem. Soc 159 (7).
6. Roth, E. P. (1999) Thermal Stability of Li-Ion Cells, United States. https://www.osti.gov/servlets/purl/14010.
7. Suurkuusk, J., Suurkuusk, M., and Vikegard, P. (2017) A multichannel microcalorimetric system: The third generation thermal activity monitor (TAM III), J. Therm. Anal. Calorim. 131.
Additional Resources
For further information, please see the corresponding video, An Overview of Isothermal Microcalorimetry in Battery R&D and QA.
Acknowledgement
This paper was written by Peter Vikegard, Ph.D., Applications Support at TA Instruments.
For more information or to request a product quote, please visit www.tainstruments.com/sales-contact to locate your local sales office information.
Click here to download the printable version of this application note.