キーワード:DSC、示差走査熱量測定、リチウムイオンバッテリー、熱暴走、カソード、アノード
TA467-JA
要約
リチウムイオンバッテリーにおける熱暴走は、安全上の重大な懸念事項です。電極材料が発熱反応を経て、温度が上昇したり反応速度が加速する場合に発生する可能性があります。反応の開始温度、機構、およびエネルギー放出がバッテリーの充電状態によって影響され得ることは周知されています。さまざまな充電状態でのカソードおよびアノード材料の熱安定性を評価するために示差走査熱量測定を用いることができます。反応の開始温度、ならびにピーク反応温度や放出エネルギーは、示差走査熱量測定(DSC)を用いて、材料をいろいろな温度について走査することによって決定することができます。このアプリケーションノートでは、リチウムニッケルマンガンコバルト(NMC)カソードおよび黒鉛アノードを評価し、カソードにおいてより高い反応エネルギーがあること、そして充電状態の低下に伴う熱安定性が向上したことを認めました。
はじめ
リチウムイオンバッテリー(LIB)は、高エネルギー密度などの好ましい特性により、さまざまな用途で広く使用されています。ただし、LIBの安全上の懸念から、バッテリー管理システムが必要です。バッテリーが過充電されたり、高温にさらされたり、短絡したりすると、熱暴走が発生する可能性があります。臨界温度に達すると放熱反応が起こり、温度が上昇し続け、それが反応速度をさらに加速させます。熱暴走中に壊滅的な劣化が発生し、有毒ガス発生やバッテリーの発火につながります。
LIBの充電状態(SOC)が熱暴走に直接影響していることは確立されています。SOCの差はバッテリーセルの熱構成を変化させます。また、電荷の低減は潜在的なエネルギー放出を低下させるため、SOCが低いバッテリーでは熱危険性が低減されます[1] [2]。バッテリーに使用される材料もまた熱暴走に影響を与えるので、さまざまな調合やSOCについて熱暴走の開始温度を理解することは熱管理の設計および熱暴走の防止において重要です。
示差走査熱量測定は、電極材料の熱流を測定するために使用することができます。さまざまな温度をスキャンすることで、熱暴走につながる可能性のある発熱反応を検出できます。材料のSOCが開始温度や放出されるエネルギー量に及ぼす影響を測定できるため、示差走査熱量測定はバッテリー安全性の評価に役立つツールとなります。
実験
異なる充電状態のバッテリーはNEI Corporation社から提供され、NMC811カソード及び黒鉛アノードを備えていました。試験の前に、バッテリーを解体し、電極をジメチルカーボネート(DMC)で洗浄して電解質を除去しました。このステップにより、電解質の分解を捉えずにカソードおよびアノード材料のみを評価する測定が保証されます[3]。電極を乾燥させた後、カソードおよびアノードの活性材料を試験のために集電体から取り外しました。充電されたサンプルと比較するために、入手したままのカソードとアノードサンプルもSOCサンプルと同じ洗浄・乾燥手順を用いて調製しました。
TA Instrumentsの示差走査熱量計(DSC)を使用して電極材料の熱流を測定しました。約5 mgのサンプルを新製品の高温高圧パン(P/N 900803.901)にロードし、5℃/分で450℃まで昇温させました。開始温度、反応熱(エンタルピー)およびピーク温度を、TRIOSソフトウェアを使用して解析しました。
結果および考察
完全に充電されたNMCカソードと黒鉛アノードの開始温度を図1に示します。第1の開始温度は、ベースラインで観察される最初の発熱シフトであり、しばしば固体電解質界面(SEI)層の初期分解に関連しており、これはその後望ましくない発熱反応を引き起こす可能性があります。アノードは82℃の低い開始温度を有し、これは一般的な黒鉛アノードにおいて典型的です。多くの場合、黒鉛アノードは80℃から120℃の間でSEIの初期分解を示します[4]。

図2で黒鉛熱流信号下方の面積がより小さいことからわかるように、黒鉛アノードは、より低い開始温度を有するにもかかわらず、全体的にNMCカソードよりもエネルギー性が低いです。この面積は反応のエンタルピーであり、熱流信号曲線を積分することによって計算すことができます。SOCが100%のNMCカソードにおいてエンタルピーは1618 J/gであり、SOCが100%の黒鉛アノードにおいてエンタルピーは345 J/gです。SOCが100%のNMCカソードでは、SOCが100%の黒鉛アノードよりも高エネルギー性の発熱反応が起きます。カソード材料の分解に関連している発熱反応は、227℃、321℃、および378℃で発生します。NMCは270℃を超えると熱的に不安定になり、酸素を放出することが知られています[5]。321℃および378℃のピークは、この熱不安定性に関連している可能性があります。完全に充電した黒鉛アノードでは、268℃ではるかに小さい発熱反応になります。カソード反応のより高いエネルギー放出により、熱暴走やバッテリー間での伝播が引き起こされる可能性が高くなります。

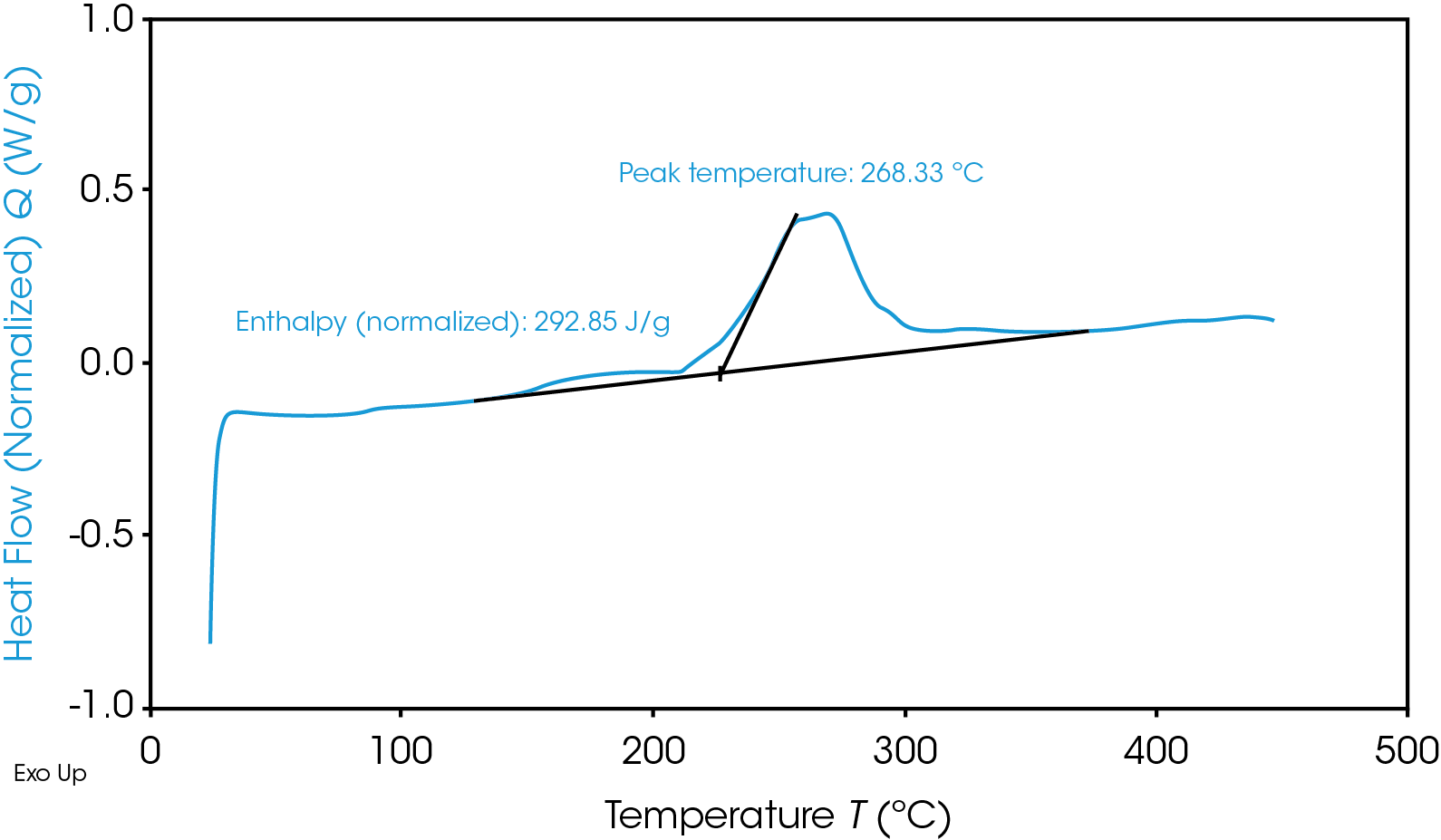
さまざまなSOCにおけるNMCカソードの熱流信号を図3に示します。SOCが低下するにつれて、熱安定性が増加しました。50%のSOCでは、主な発熱イベントピークは378℃からより高い416℃の温度にシフトし、100%のSOCの場合よりも少ないエネルギーが放出されました。充電されていないカソード材料は発熱反応を起こしませんでした。
予想通りアノードは、図4に示されているように、同様の傾向に従いました。アノードのSOCが減少すると、対応する熱流も減少しました。放熱反応は、SOCが50%の場合、SOCが100%の場合よりも少ないエネルギーを放出しました。さまざまなSOCにおける両方の材料の単位質量当たりのエンタルピーを表1に示します。
Table 1. Enthalpy of reactions at various SOC for the NMC cathode and graphite anode
Enthalpy (J/g) | NMCカソード | 黒鉛アノード |
---|---|---|
0% SOC | 72.1 | 49.8 |
50% SOC | 625 | 216 |
100% SOC | 1618 | 345 |

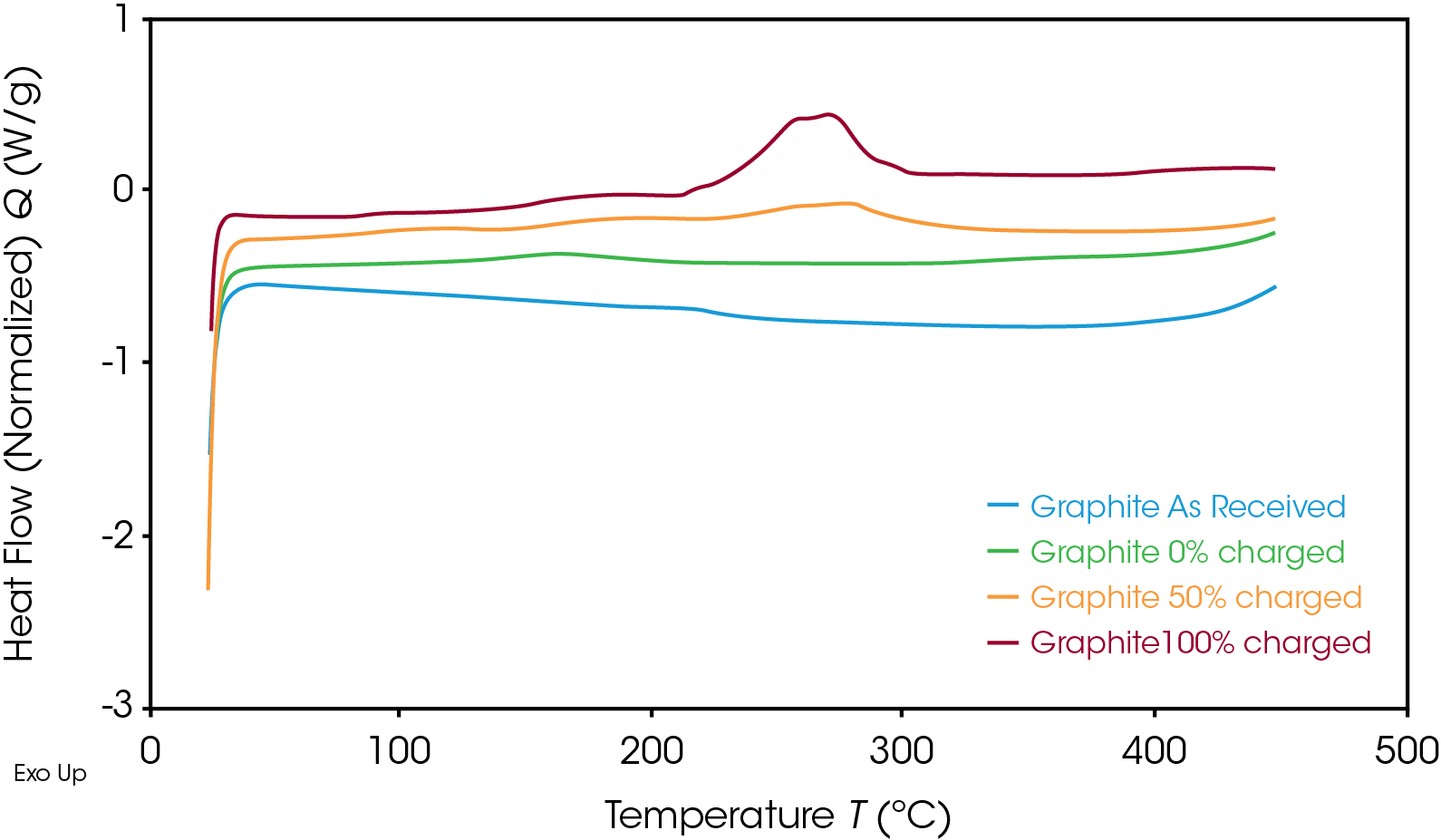
充電されていない場合、黒鉛アノードとNMCカソードの反応エンタルピーは類似しています。しかしながら、このバッテリーについては、充電された黒鉛アノードで放出されるエネルギーは、充電されたNMCカソードのエネルギーよりも有意に低く、カソードが熱暴走を引き起こすリスクが高いことを示しています。このバッテリーの完全充電状態のアノードと比較して、完全充電状態のカソードは3倍超のエネルギーを放出します。このようなエネルギーの放出が、熱暴走につながるバッテリー内の反応を駆動する可能性は高いです。両方の電極を研究して、どの材料がより高い危険因子を持ち、どの温度を避ける必要があるかを判断することが重要です。危険な劣化や熱不安定性を避けるために、完全充電状態のバッテリーを開始温度未満にとどめる必要があります。DSCの結果は、バッテリー材料の安全パラメータの理解を介して、熱管理システムの設計に役立ちます。
おわりに
DSCは、熱暴走に関する電極材料の安全解析に使用できます。熱暴走につながる可能性のある反応の温度、ならびに分解反応中に放出されるエネルギーの量を決定することができます。異なるSOC(100%、50%、0%)の電極を分析し、SOCが100%のNMCカソードは、熱暴走およびバッテリー間の伝播のリスクが最も高いことがわかりました。SOCが50%に低下すると、NMCは熱的に安定化し、発熱反応はより高い温度にシフトしました。黒鉛アノードは、SOCに関して同じ傾向に従っていながら、カソードよりもはるかに少ないエネルギーを放出しました。
参考文献
- L. Torres-Castro, A. Kurzawski, J. Hewson and J. Lamb, “Passive mitgation of cascading propagation in multi-cell lithium ion batteries,” Journal of the Electrochemical Society, vol. 167, no. 9, 2020.
- A. W. Golubkov, S. Scheikl, R. Planteu, G. Voitic, H. Wiltsche, C. Stangl, G. Fauler, A. Thaler and V. Hacker, “Thermal runaway of commercial 18650 Li-ion batteries with LFP and NCA cathodes- impact of state of charge and overcharge,” RSC Advances, vol. 5, pp. 57171-57186, 2015.
- Z. Zhang, D. Fouchard and J. R. Rea, “Differential scanning calorimetry material studies: implications for the safety of lithium-ion cells,” Journal of Power Sources, vol. 70, pp. 16-20, 1998.
- X. Feng, M. Ouyang, X. Liu, L. Lu, Y. Xia and X. He, “Thermal runaway mechanism of lithium ion battery for electric vehicles :A review,” Energy Storage Materials, vol. 10, pp. 246-267, 2018.
- K. Kim, D. Kam, C. C. Nguyen, S.-W. Song and R. Kostecki, “Study on the Dominant Film-Forming Site Among Components of Li(Ni1/3Co1/3Mn1/3)O2 Cathode in Li-ion Batteries,” Bulletin of the Korean Chemical Society, vol. 32, no. 8, 2011.
謝辞
このアプリケーションノートは、NEI Corporation(ニュージャージー州サマセット)とTA Instrumentsのコラボレーションによるものです。TA Instrumentsの新市場展開科学リーダーであるJennifer Vail博士とHang Lau博士によって作成されました。
このアプリケーションノートの印刷用バージョンをダウンロードするにはここをクリックしてください。